Review Article
A Gateway to Metal Resistance: Bacterial Response to Heavy Metal Toxicity in the Biological Environment
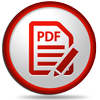
Loai Aljerf1* and Nuha AlMasri2
1Department of Basic Sciences, Faculty of Dental Medicine, Damascus University, Mazzeh Highway, Almazzeh, Damascus, Syria
2Department of Chemistry, Faculty of Medicine, Syrian Private University, Damascus, Syria
*Address for Correspondence: Loai Aljerf, Department of Basic Sciences, Faculty of Dental Medicine, Damascus University, Mazzeh Highway, Almazzeh, Damascus, Syria, Tel: +963-93 34 46 993; Email: [email protected]; [email protected]
Dates: Submitted: 21 June 2018; Approved: 01 September 2018; Published: 03 September 2018
How to cite this article: Aljerf L, AlMasri N. A Gateway to Metal Resistance: Bacterial Response to Heavy Metal Toxicity in the Biological Environment. Ann Adv Chem. 2018; 2: 032-044. DOI: 10.29328/journal.aac.1001012
Copyright License: © 2018 Aljerf L, et al. This is an open access article distributed under the Creative Commons Attribution License, which permits unrestricted use, distribution, and reproduction in any medium, provided the original work is properly cited.
Keywords: Heavy metal; Phytochemical agents; Microorganism; Environment; Impermeability; Resistance transfer factors; Chelators
Abstract
Heavy metals and metalloids are dangerous because they have the tendency to bioaccumulate in biological organisms over a period of time. However, it is conceived that a number of phytochemical agents as well microorganism can act as heavy metal removing agent both from human beings and the environment surrounding. For instance, microbes are used for the removal of heavy metals from the water bodies including bacteria, fungi, algae and yeast. This review shows that bacteria can play an important role in understanding the uptake and potential removal behaviour of heavy metal ions. The bacteria are chosen based on their resistance to heavy metals (incl. their toxicities) and capacity of adsorbing them. Due to specific resistance transfer factors, cell impermeability is drastically inhibited by several ion (i.e. mercury, cadmium, cobalt, copper, arsenic) forms. Between these elements, free-ion cadmium and copper concentrations in the biological medium provide more accurate determination of metal concentrations that affect the bacteria, than with most of the other existing media. Metal toxicity is usually assessed by using appropriate metal ion chelators and adjusting pH factor. Bacteria and metals in the ecosystem can form synergistic or antagonistic relationships, supplying each other with nutrients or energy sources, or producing toxins to reduce growth and competition for limiting nutritional elements. Thus, this relation may present a more sustainable approach for the restoration of contaminated sources.
Introduction
The elements which are known collectively as the “heavy metals” are a fairly ill-defined group. Generally they include many of the transition series of metals and some of the metals and metalloids in groups IIIB, IVB, VB and VIB of the periodic table. Although many of them are micro-nutrients, they are primarily of interest because of their toxic properties to all forms of life [1].
Probably the most important feature which distinguishes the heavy metals from other toxic pollutants is that they are not biodegradable, and having entered the environment where their potential toxicity is controlled to a great extent by biological and geochemical factors [2]. The toxicity of heavy metals to plants [3,4] and animals [5] is well known. It is primarily the avidity of heavy metals for natural metal-binding agents which determine their toxicity. They may cause disruption of enzyme structure and function by binding with thiol and other groups on protein molecules which may replace metals naturally occurring in enzyme prosthetic groups [6]. Metals have also been shown to bind with and disrupt deoxyribonucleic acid (DNA) [5]. The bioaccumulation of some metals is an important aspect of their toxicity [7,8] which may result in the appearance of symptoms after prolonged exposure; their accumulation may also lead to mobilisation through food chains [9] with possible effects on higher organisms.
There is convincing evidence that some metals, notably chromium and nickel, can cause cancer, and tentative evidence for many more [10]. The form of metals may increase their toxicity: dimethyl mercury and tetraethyl lead are particularly dangerous since they may easily enter the body and remain there as a result of their high lipid solubility [4,11]. Volatile metals and their compounds may also be dangerous since they may enter the body through the lungs [12], while organomercurials may pass through the placenta [13].
As a group, the bacteria are important agents in determining the form and distribution of metals in the environment. They play a major part in the modification, activation and detoxification of heavy metals [14]. However, they may themselves be subject to metal toxicity. This is of importance in some key processes, such as biological waste treatment [15,16] and also in the field of medicine [17].
Heavy metal toxicity to bacteria
Several workers have studied the toxicity of heavy metals to pure bacterial cultures. Although it is apparent that different species have different responses [18], some trends are evident. Waturangi et al. [19], found for the species they tested, that actinomycetes were more tolerant to cadmium than Gram negative bacteria, which were more tolerant than Gram positive bacteria. These differences may be due to the different biochemical and morphological characteristics of the groups. This may be reflected in the distribution of metals in cellular fractions. During an investigation of the effects of inorganic lead salts on Azotobacter sp. and Micrococcus luteus [20], 37.6% of the lead immobilised by Azotobacter sp. was found in the cell wall, compared to only 9.5% of that immobilised by M. luteus.
The outer layers of cells are probably very important in determining how much of a metal penetrates the cytoplasm. Of the lead abstracted from a medium containing 600 mg/L lead bromide or lead nitrate by M. luteus, 75 to 82% was found in lipid extracts of the cells [21]. Analysis showed that no specific plumbated lipids were present, thus it appears that only a natural mixture of cell lipids had the capacity for lead retention [22].
The lead caused structural inconsistency of the cytoplasmic membrane, and attempts to prepare protoplasts by treatment of cells with lysozyme often resulted in protoplasmic lysis [23]. Some other bacteria have been shown to undergo plasmolysis and changes in mesosomal structure indicative of membrane disruption [24]. Treatment of the extracted lipid fraction with tris (hydroxymethyl) aminomethane and ethylenediaminetetracetate (EDTA) or reduction of thiol groups with p-chloromercuric phenylsulphonic acid had little effect on lead retention [25].
A fairly common feature of the effects of sub-lethal concentrations of metals on bacteria is retardation of the onset of growth. Methylmercury acetate extended the lag phase of cultures of Rhodopseudomonas capsulata, but cultures which did begin to grow reached limiting cell densities similar to that of a control [26]. Cadmium had the effect of extending the lag phase of cultures of Escherichia coli, but normal proliferation was observed at the end of the lag phase [27]. During lag phase 95% of cells lost viability, and various structural abnormalities were observed, but by the middle of the lag phase cells had resumed normal morphology. Extension of the lag phase by mercuric chloride was also accompanied by a decrease in viability of R.capsulata although the turbidity remained the same [28]. This effect would therefore not be observed if culture turbidity was used solely as a measure of growth. It was suggested that growth would only occur when the available metal had been reduced to a threshold concentration, and that this may have been brought about by cell lysis during the decrease in viability [29]. However, in the case of E. coli, Mokkapati et al. [30] have suggested that the cells develop some mechanism of molecular accommodation during this phase. This concept is supported by the changes in cellular distribution observed during growth.
Species specific sensitivity to heavy metals may have pronounced effects on natural microbial populations. Although effects on total viable counts may be minimal, the sensitivity of one type or group of organisms may result in an appreciable change in the behaviour of the population [31]. Singleton and Guthrie [32] studied the effects of added copper and mercury on aerobic, heterotrophic bacterial populations of two aquatic systems. Although 2 mg/L copper or mg/L mercury caused an increase in the total number of colony forming units, the colony type diversity decreased in both systems. Additions of copper and mercury also stimulated an increase in uptake of a number of other elements [33]. Albright and Wilson [34] found that the heterotrophic activity (based on uptake and mineralisation of 14Cglucose) of a natural population decreased on addition of 10 µg/L copper and 50 µg/L mercury, but the numbers of viable heterotrophic bacteria were unchanged. However, the addition of 10 and 50 µg/L copper to two marine ecosystems led to a marked increase in the relative numbers and activity of heterotrophs [35].
Biological waste treatment processes employ natural microbial populations to treat organic wastes. Bacterial sludge has an ability to remove metals from solution [36] but they are also subject to the toxic effects of such metals. The toxic effects of metals include deflocculation [37] and a decrease in the respiratory activity of the sludge [38] leading to poor effluent quality. Anaerobic digestion is also susceptible to metal toxicity [39].
Metal form and toxicity
Several factors affect the form of metals, and thus their potential toxicity. These include pH, concentration of chelating agents, concentration of inorganic anions and competition from other cations. Shi et al. [40] found that the toxicity of cadmium to some bacteria was enhanced at alkaline pH, while for others it was independent of pH. It would have been virtually impossible to determine metal form because of the complex nature of the growth medium used. Precipitation of a metal may cause a reduction in toxicity by preventing its access to bacteria. It was found that inhibitory concentrations of copper in a seawater medium had no effect on the growth of E. coli, when added to the medium before autoclaving [41]; seawater precipitates considerably on heating. Soltani and Shaheli [17] suggested that precipitated metals may exert an effect on bacteria, but this was based on the assumption that metals retained in solution by chelating agents would be available to exert a toxic effect on the bacteria.
A number of natural and synthetic chelating agents can reduce the toxicity of heavy metals. Cysteine has been shown to protect bacteria against the toxicity of methyl mercury acetate [42], EDTA abolished the toxic effect of added copper to Nitrosomonas [43] and cysteine hydrochloride promoted more rapid onset of growth in cultures of E. coli retarded by copper [44]. Nitrilotriacetate and citrate have also been shown to protect fish from copper and zinc toxicities [45]. Cadmium sensitive strains of Staphylococcus aureus pre-treated with cysteine were protected from penetration of the metal into the cells, but treatment after metal uptake had occurred did not lead to release of cadmium from the cells [46]. Clay minerals have been shown to influence the toxicity of cadmium to bacteria. Montmorillonite, and to a lesser extent, kaolinite, decreased the inhibitory effects of cadmium [47]. The greater protective effect of montmorillonite was correlated with its higher cation-exchange capacity (CEC). However, clays homoionic to cadmium (i.e. already saturated with the metal) enhanced the toxicity of exogenous cadmium, montmorillonite again having the greatest effect due to its higher CEC [48].
Metals may also have their toxicity reduced by common components of nutrient media. Leitao and Sa-Correia [49] found that growth of Pseudomonas aeruginosa in the presence of inhibitory concentrations of copper was improved by increasing the concentrations of nutrients in the medium. It has been demonstrated that three common agar media neutralised to differing degrees the bacteriostatic effects of silver [50]. These examples indicate that media constituents should be taken into account where studying metal toxicity to bacteria and that such study may not reflect the real situation in a natural environment.
Another aspect of metal form which may influence toxicity is the valence of metal ions and their compounds. Panda and Sarkar [51] found Cr3+ to be less toxic to K. aerogenes than CrO42-. A 24% reduction in glucose oxidation by the bacteria in a laboratory scale continuous flow reactor was induced by 12.4 mg/L Cr3+, whereas only 4.9 mg/L CrO42- caused a reduction of 45% [52]. Both Cr6+ and Cr0 are known to induce cancer in experimental animals, but there is no evidence that Cr3+ has a similar effect [53]. A mixed microbial population from activated sludge was inhibited during lag phase of growth to a greater extent by Cd2+ than Cd(CN)42-, but there were no differences between them in the effects on substrate utilisation [54]. Other metals may have similar properties. The fact that, a strain of S. aureus has been found to possess a plasmid carrying separate resistance determinants for arsenate and arsenite [55] suggests that the properties of arsenic in these two anionic species axe significantly different, and this may influence their toxicity.
Obviously, it is unlikely that in a natural environment, bacteria are exposed to the effects of a single metal. Synergism or antagonism may occur with mixtures of metals. Babai [56] found that growth of E. coli was inhibited at very low concentrations of nickel, cobalt, cadmium, zinc and manganese when magnesium was not present in the medium. Their toxicity was markedly reduced in the presence of magnesium. Magnesium had similar effects on the toxicity of nickel and cobalt to Aerobacter aerogenes, and it was found that higher levels of magnesium reduced the amounts of these metals bound by the cell [57]. Tsai [58], in a study of chromium and copper sensitivity in K. aerogenes, found both have synergistic and antagonistic effects. Very low concentrations of Cd2+ or Zn2+ potentiated the lethal action of Cu2+, mixtures of Cu2+ and CrO42- gave an additive response, and mixtures of Cd2+ with CrO42- were antagonistic.
The antimicrobial activity of some toxic compounds may be increased by heavy metals. Heavy metal derivatives of sulphonamide drugs were found to have greater antimicrobial activity than the parent compounds [59]. Copper ions were shown to increase the growth inhibitory effect of 2,2’-bipyridyls on mycoplasmas [60]. A strong inhibitory effect on Mycoplasma gallisepticum was noted only in the presence of exogenous Cu2+. The tetrahedral complexes formed by Cu2+ (and Zn2+ and Cd2+, which had lower activity) with 2,2’-bipyridyls are highly stable and lipophilic, which probably allows them easy access to the cell [61]. Other examples of augmentation of the toxic effect of metals by chelation include the formation of a lethal complex between 8-hydroxylquinoline and a metal, even when the concentration of the metal itself (for example iron) is not toxic [62]. The toxic action of the 8-hydroxylquinoline iron complex can be antagonised by cadmium, cobalt, zinc and nickel [63].
Obviously, some metals are intrinsically more toxic than others. Since heavy metals act primarily as a result of their affinity for chelating agents, an assessment of the degree of affinity may indicate the comparative toxicity of a metal. Dipu et al. [64] has stated that most chelating agents show approximately the same order of preference for metals. For decreasing order of affinity this series is:
Fe3+, Hg2+ > Cu2+, A13+ > Ni2+, pb2+ > Co2+, Zn2+ > Fe2+, Cd2+ > Mn2+ > Mg2+ > Ca2+ > Li+ > Na+ > K+.
However, there are many cases of departure from this series. Madoni et al. [65] found that Hg2+ was less toxic to heterotrophs than Cu2+ and Ni2+, and lead was found to be adsorbed by activated sludge more efficiently than copper and cadmium. It is apparent, therefore, that although a general trend may be evident; this alone has limited value in the estimation of toxicity.
Bacteria and metal form
Bacteria can, to some extent, determine the forms of heavy metals to which they are exposed. This may occur by transformation to less toxic forms, immobilisation by uptake or mobilisation from sinks. The mechanisms involved may be specific for the metal or non-specific.
Immobilisation of metals by bacteria is an important feature of the activated sludge process [66]. Gu et al. [67], have suggested that it may be possible to develop a practical microbiological process to remove metals from water. They evaluated the potential of Zoogloea ramigera cellflocs for removal of cadmium and mercury from solution, and found that cells grown in an arginine-glucose medium accumulated considerably less mercury than cells cultivated in trypticase soy broth. The possibility that affinity for metals is influenced by nutrient source and metabolism has also been considered by Abdulaziz et al. [68]. Cell-associated radioactive zinc in growing cultures of Z. ramigera was found to decrease between the fifth and seventh day of growth. This release from the cell floc may indicate a conversion from one metabolic activity to another.
Although it is unlikely that insoluble metal precipitates could exert a toxic effect on bacteria, they may be mobilised by the bacteria themselves. Chen and Weimer [69] showed that the solubility of copper in the absence of bacteria was increased three fold in their presence. It was suggested that this was due to the production of chelating agents by the bacteria. However, the distinction made between soluble and insoluble metal here may be misleading. Metals may form many different species, and it is probably an over simplification to suggest that only two classes of metal species (i.e. precipitated and those apparently in solution) are significantly different in their toxic effects. Pseudomonas fluorescens may mobilise and accumulate metals from sediment and river water [70]. A multicompartment system of bacteria accumulated about twenty times more than the sediment. The bacteria were also able to remove mercury from the sediment at a rate much higher than the normal rate of adsorption. This may be significant since sediment acts well as a sink, and in highly polluted waters may have loads of greater than 9000 mg/L of mercury [71].
Some microorganisms are thought to guard against mineral deficiencies by providing themselves with chelating agents which may aid in the absorption and conservation of essential trace elements. Compounds may be released into the medium in conditions of iron deficiency. Upon re-entry of the iron complex into the cell, the compounds are broken down enzymatically to release the iron [72]. Although synthesis of iron-fixing compounds usually only occurs in conditions of iron deficiency, the complexation of iron by EDTA and α,α’-dipyridyl in a medium containing plentiful amounts has been shown to reduce the free concentration of the metal such that synthesis of iron-fixing catechols by K. aerogenes was induced [73]. It is probable that uptake of the complexes is associated with specific transmembrane pores, since acquisition of a new transport system by strains of E. coli, originally deficient in iron-uptake capability, has been associated with the loss of two major outer membrane proteins [74].
Bacteria may produce more or less toxic forms of metals by transformation [75]. Some metals may be methylated by bacteria [76] and other organisms [77]. Mercury may be methylated by the transfer of methyl groups from methylcobalamine in extracts of ethanogenic bacteria [76] and microorganisms present in lake sediment can transform inorganic lead compounds into volatile tetramethyl lead in anaerobic conditions [78]. Kobza [79] has suggested that it may be possible to predict which metals could be methylated. Methylcobalamine does not transfer methyl groups to lead, cadmium and zinc, but may to tin, palladium, platinum, gold and thallium. The isolation of tetramethyl lead [4,80] suggests, therefore, that other mechanisms of methylation may occur. The stability of alkylmercurials may result in mercury releases into the environment being more dangerous than other metals, whose alkyls are relatively unstable.
Although methylation is known to occur in aquatic environments, the failure by many workers to isolate methyl mercury maybe due in part to the presence of bacteria capable of degrading methyl mercury [81]. Aerobic incubation of sediment containing Hg2+ gave rise to the production of methyl mercury during the first day, but this was followed by a rapid decrease in methyl mercury concentration concomitant with a rapid increase in the amount of volatile Hg0 produced [82]. Wright and Hamilton [83] stated that, for a given culture, neither the type of substrate its concentration nor its rate of addition, had any effect on the rate of aerobic decomposition of methyl mercury and they suggested that an actively growing population is not necessary for decomposition, and that an enzyme system may be involved.
It was found that the induction period before the onset of degradation was decreased by pre-incubation of river sediment in increasing concentrations of methyl mercury, and that the mineralising activity appeared to be lost on prolonged incubation in the absence of mercury [84]. The authors suggested that preincubation in the presence of methyl mercury favours the development of a sub-population capable of degradation, while in non-preincubated cultures that part of the population having degradative activity was present at such a low level that the rate of mineralisation was below the detection limit initially.
The decomposition of phenylmercuric acetate to benzene and Hg0 by a Pseudomonas sp. from soil [85] was found to be mediated by an enzyme designated metallic mercury-releasing enzyme (MMR-enz). The enzyme was induced by phenylmercuric acetate, p-chloromercuric benzoate, sodium ethyl mercuric thiosalicylate, mercuric chloride and metallic mercury; D-glucose: NAD oxidoreductase (or L-arabinose: NADP oxidoreductase) and cytochrome C, were required for activity [86]. The phenylmercuric acetate was only degraded once it had entered the cell. A large amount of radioactive phenylmercuric acetate was absorbed by the cells, and this disappeared during the logarithmic phase of growth although hardly any free phenylmercuric acetate disappeared from solution [87].
Oxidation of Hg0 by several bacterial species has also been observed. Graham et al. [88] studied the stability of Hg0 in two culture media. In a glucose medium it was stable, but in yeast extract medium it was slowly oxidised. Growth of the bacteria significantly increased the oxidation of Hg0, as did a sterile filtrate from a 48 h culture of Bacillus megaterium. The conversion of Hg2+ to Hg0 may be regarded as a detoxification mechanism since Hg0 is more readily lost from the aquatic environment. In fact, all interconversions are cyclic, and unless disruption occurs, equilibrium will be maintained [89].
Resistance
Two types of resistance are discussed here: non-specific resistance arising from differences in physiological state of the organism, and inheritable specific resistance factors for particular heavy metals. Both may be important for different reasons. Impermeability or detoxifications by chelation are both non-specific mechanisms of resistance. The formation of a complex or chelate with bacterial extracellular polymers is an important aspect of metal removal from wastewaters. Krul [90] studied the uptake of metals by Z. ramigera strain 115, which produces a gelatinous matrix, and strain I-16-M. Strain 115 showed a high affinity for cobalt, copper and iron, took up about twice as much metal as strain I-16-M. Work with capsulated and non-capsulated strains of K. aerogenes showed that the capsulated strain survived better in the presence of copper and cadmium (10 mg/L) [91]. Extracellular polymer extracted from one strain, when mixed with the metals, exerted a protective effect on the non-capsulated strain. Analysis by ion-selective electrodes (ISE) showed that, at the concentrations used, the extracted extracellular polysaccharide complexed 54% and 9% of the copper and cadmium respectively [91].
Brandt et al. [92], found that periphytic Pseudomonas spp. produced significant quantities of extracellular polymers, and that growth in the presence of copper did not stimulate that production. Of the copper taken up by the cells, most was found immobilised in the polymer layer, and very little gained access to the cytoplasm. An Azotobacter sp. was found to be more efficient in immobilisation of lead than M. luteus [93], this probably being due to the large quantity of capsular material surrounding the cells (M. luteus is non-capsulated). The relative sensitivity of Nitrosomonas to chromate compared with Nitrobacter may be due to differences in intracytoplasmic membranes [94]. These encircle the entire cell of Nitrosomonas, but are restricted to lesser areas of Nitrobacter.
The density of a bacterial culture or population may also influence the toxicity of heavy metals. The inhibitory effects of copper on the growth of bacterial cultures can be eliminated by the addition of more living or dead cells [95]. The toxic effects of metals on the activated sludge process may be alleviated by increasing the suspended solids (biomass) concentration [96]. Resistant species which can accumulate metals may exert a protective effect on sensitive species in the same system by removing the metal from the system. A strain of E. coli which was twenty times more resistant to mercuric chloride and merbromin than S. aureus caused a decrease of 50% in sensitivity of the latter when suspensions of the two were mixed [97]. E. coli suspensions took up about five times as much mercury than S. aureus, and the protective effect was amplified by the secretion by E. coli of glutathione into the medium, which when added to mercury-inhibited cultures of S. aureus could relieve the toxic effects of the metal.
The introduction of antibiotics in recent years has led to the appearance of specific resistance factors to these agents in a number of bacteria. Similarly, resistance factors to a number of heavy metals have occurred. These factors are determined by extrachromosomal genetic material called plasmids. These genetic elements, which are self-replicating, may be transferred to bacteria of the same and similar species; hence they are often known as Resistance Transfer Factors (RTFs) [98].
Specific resistances to metals, which are often found linked to antibiotic resistances, can make the bacteria which possess them resistant to as much as one thousand times the concentration causing inhibition of sensitive strains. In contrast, a plasmid carrying determinants for increased sensitivity to mercuric chloride and cobalt chloride in some strains of E. coli K-12 has been discovered [99].
Götz et al. [100], found that penicillinase plasmids of S. aureus carried determinants for resistance to arsenate, arsenite, lead, cadmium, mercuric and bismuth ions. Resistances to antimony and zinc were also found, but these were not distinguishable from resistance to arsenite and cadmium respectively. This is probably because the chemistry of cadmium and zinc, at least, are essentially homologous [101]. Resistance factors for organomercurials have also been discovered [102]. The mechanisms of resistance to mercury and cadmium mediated by the penicillinase plasmid of S. aureus were found to be entirely different [100]. The resistance to mercury was probably inducible since during the lag phase of growth cells lost viability followed by a gradual increase to the level of the control. The resistance to cadmium was probably due to an impermeability barrier [103], which is specific since the uptake of other essential metals (magnesium and calcium) was not affected [104]. An impermeability barrier to Co2+ has been observed in a resistant strain of E. coli B. Electrophoretic analysis of membrane proteins showed slight differences between the strains, suggesting a modification in the specific membrane transport system for cobalt [105].
The possession of drug resistance RTFs by coliform bacteria has lead to concern about water quality standards and public health [106]. However, drug resistances have been found associated with heavy metal resistance factors in samples from sewage [107], polluted sites [108] and clinical sources [109,110]. In fact, resistance to heavy metals is virtually always found associated with resistance to antibiotics [111,112] and the frequency of heavy metal resistance is often the same as or higher than the frequency of drug resistance [113]. Resistant Bacillus populations have a greater frequency in sites polluted by mercury containing sewage sludge than in unpolluted sites, and ampicillin resistance follows the same pattern [114-116]. The fact that Bacillus spp. with the combined resistance were six times more frequent in the sludge dump site, suggested that resistances may be co-selected for, and that metal pollution may exert a selection pressure for antibiotic resistances, thus further increasing their clinical importance.
Conclusion
Bacteria are generally the first organisms to be affected by discharges of heavy metals into the environment. Each of the wide range of species may be affected in a different way, and although low concentrations of metals may have only imperceptible effects on total viable counts of natural populations, the balance of species, and thus the metabolic characteristics of the population, may be drastically affected.
The major factor determining the toxicity of heavy metals to bacteria is probably the extent to which they penetrate the cytoplasm. Many bacteria appear to have the capacity for adsorbing metals in the outer layers of the cell. The synthesis of capsules by some species is important both in terms of resistance to toxicity and in detoxifying the environment by removal of metals from solution. Metals may also be prevented from entering the cell by the formation of complexes or chelates with several metal-binding agents. Although these agents may serve to protect microbial populations, their influence on metal uptake may have serious effects in waste-treatment processes where immobilisation of metals by the biomass is the most important mechanism of metal removal.
Apart from their role in immobilising heavy metals, bacteria may affect their environmental distribution in other ways. Some metals are particularly susceptible to alkylation by some bacteria, and subsequent dealkylation by others. Metals may also be mobilised from sinks either by accumulation or by complexation with excreted metabolic products.
In recent years the increasing frequency of antibiotic resistance in bacteria has been correlated with specific determinants for heavy metal resistance. Although, bacterial populations having resistance to high concentrations of heavy metals may be advantageous, the association with antibiotic resistance may lead to co-selection, thus enhancing the clinical importance of antibiotic resistance.
References
- Jung H. Nutrients and heavy metals contamination in an urban estuary of northern New Jersey. Geosciences. 2017; 7: 108. Ref.: https://tinyurl.com/ycnj3936
- Aljerf L, Choukaife AE. Review: Assessment of the doable utilisation of dendrochronology as an element tracer technology in soils artificially contaminated with heavy metals. Biodiversity International Journal. 2018; 2: 1-8. Ref.: https://tinyurl.com/yc3hkbnl
- Mustafa G, Komatsu S. Toxicity of heavy metals and metal-containing nanoparticles on plants. Biochim Biophys Acta. 2016; 1864: 932-944. Ref.: https://tinyurl.com/y8zo7dbq
- Aljerf L, Al Masri N. Mercury toxicity: ecological features of organic phase of mercury in biota-Part I. Archives of Organic and Inorganic Chemical Sciences. 2018; 3: 1-8. Ref.: https://tinyurl.com/yazn237c
- Jadoon S, Malik A. DNA damage by heavy metals in animals and human beings: an overview. Biochem Pharmacol. 2017; 6: 1-8. Ref.: https://tinyurl.com/yartpqd9
- Warburg O. Heavy metal prosthetic groups and enzyme action. Soil Sci. 1950; 70: 166. Ref.: https://tinyurl.com/ybqu4znr
- Lalotra P. Bioaccumulation of heavy metals in the sporocarps of some wild mushrooms. Curr Res Environ Appl Mycol J Fungal Biol. 2016; 6: 159-165. Ref.: https://tinyurl.com/y7733ojc
- Aljerf L. Advanced highly polluted rainwater treatment process. Journal of Urban and Environmental Engineering. 2018.
- Stasinos S, Nasopoulou C, Tsikrika C, Zabetakis I. The bioaccumulation and physiological effects of heavy metals in carrots, onions, and potatoes and dietary implications for Cr and Ni: A review. J Food Sci. 2014; 79: 765-780. Ref.: https://tinyurl.com/ya4cegqp
- Shah SA. Trace minerals and heavy metals: implications in prostate cancer. Bangl J Med Biochem. 2017; 8: 27. Ref.: https://tinyurl.com/ybtxln4j
- Bjørklund G, Mutter J, Aaseth J. Metal chelators and neurotoxicity: lead, mercury, and arsenic. Arch Toxicol. 2017; 91: 3787-3797. Ref.: https://tinyurl.com/y9dlr5u2
- Feingold A. The elimination of volatile substances from the lungs. Int Anesthesiol Clin. 1977; 15: 153-168. Ref.: https://tinyurl.com/ydhge79v
- Sato Y. The pathological findings of placenta with neonatal placenta. Placenta. 2017; 59: 169. Ref.: https://tinyurl.com/yc7oneo3
- De J, Ramaiah N, Vardanyan L. Detoxification of toxic heavy metals by marine bacteria highly resistant to mercury. Mar Biotechnol. 2008; 10: 471-477. Ref.: https://tinyurl.com/y77bkov7
- Robinson T. Removal of toxic metals during biological treatment of landfill leachates. Waste Manage. 2017; 63: 299-309. Ref.: https://tinyurl.com/ybcsnoy8
- Aljerf L. High-efficiency extraction of bromocresol purple dye and heavy metals as chromium from industrial effluent by adsorption onto a modified surface of zeolite: kinetics and equilibrium study. Journal of Environmental Management. 2018. Ref.: https://tinyurl.com/y7zos28k
- Soltani N, Shaheli M. Cow milk contamination with heavy metals (mercury and lead) and the possibility of heavy metals disintegration by the human intestinal bacteria. J Med Microbiol Diag. 2017; 6: 267. Ref.: https://tinyurl.com/yazt4xew
- Mathew R, College SM, Krishnaswamy VG. Remediation of mixed heavy metals using acido-tolerant bacterial co-cultures. Int J Agric Environ Sci. 2017; 4: 43-52. Ref.: https://tinyurl.com/ybjyax2w
- Waturangi DE, Rahayu BS, Lalu KY, Mulyono N. Characterization of bioactive compound from actinomycetes for antibiofilm activity against Gram-negative and Gram-positive bacteria. Malays J Microbiol. 2016; 12: 291-299. Ref.: https://tinyurl.com/y954g78q
- Puyen ZM, Villagrasa E, Maldonado J, Diestra E, Esteve I, et al. Biosorption of lead and copper by heavy-metal tolerant Micrococcus luteus DE2008. Bioresour Technol. 2012; 126: 233-237. Ref.: https://tinyurl.com/ycsqrvno
- Barrow W, Himmel M, Squire PG, Tornabene TG. Evidence for alteration of the membrane-bound ribosomes in Micrococcus luteus cells exposed to lead. Chem Biol Interact. 1978; 23: 387-397. Ref.: https://tinyurl.com/y9vj62hn
- Bishop R. Bacterial lipids. Biochim Biophys Acta. 2016; 1862: 1285-1286. Ref.: https://tinyurl.com/ydhs6r97
- Gautam S, Sood NK, Gupta K. Aberrant cytoplasmic accumulation of retinoblastoma protein in basal cells may lead to increased survival in malignant canine mammary tumours. Vet Med. 2018; 59: 76-80. Ref.: https://tinyurl.com/ybgurms5
- Owen P, Salton MRJ. Isolation and characterization of a mannan from mesosomal membrane vesicles of Micrococcus lysodeikticus. Biochim Biophys Acta 1975; 406: 214-234. Ref.: https://tinyurl.com/yb3aucpg
- Saxena G, Flora SJS. Lead-induced oxidative stress and hematological alterations and their response to combined administration of calcium disodium EDTA with a thiol chelator in rats. J Biochem Mol Toxicol. 2004; 18: 221-233. Ref.: https://tinyurl.com/ydx8su6m
- Nielsen AM, Sojka GA. Photoheterotrophic utilization of acetate by the wild type and an acetate-adapted mutant of Rhodopseudomonas capsulata. Arch Microbiol. 1979; 120: 39-42. Ref.: https://tinyurl.com/yd2d5j4j
- Jaiganesh T, Rani JDV, Girigoswami A. Spectroscopically characterized cadmium sulfide quantum dots lengthening the lag phase of Escherichia coli growth. Spectrochim Acta A. 2012; 92: 29-32. Ref.: https://tinyurl.com/y7xsknvu
- Parran DK. Effects of methylmercury and mercuric Chloride on differentiation and cell viability in PC12 cells. Toxicol Sci. 2001; 59: 278-290. Ref.: https://tinyurl.com/y82hp9a4
- Kato F, Tanaka M, Nakamura K. Rapid fluorometric assay for cell viability and cell growth using nucleic acid staining and cell lysis agents. Toxicol In Vitro. 1999; 13: 923-929. Ref.: https://tinyurl.com/ycdm4erf
- Mokkapati SK, de Henestrosa ARF, Bhagwat AS. Escherichia coli DNA glycosylase Mug: a growth-regulated enzyme required for mutation avoidance in stationary-phase cells. Mol Microbiol. 2008; 41: 1101-1111. Ref.: https://tinyurl.com/yaadvf7l
- Cheng TC. In vivo effects of heavy metals on cellular defense mechanisms of Crassostrea virginica: Total and differential cell counts. J Invertebr Pathol. 1988; 51: 207-214. Ref.: https://tinyurl.com/y8cm89mq
- Singleton FL, Guthrie RK. Aquatic bacterial populations and heavy metals-I. Composition of aquatic bacteria in the presence of copper and mercury salts. Water Res. 1977; 11: 639-642. Ref.: https://tinyurl.com/y7k45ttd
- Bradberry SM. Metals (cobalt, copper, lead, mercury). Medicine. 2016; 44: 182-184. Ref.: https://tinyurl.com/ybfj8flz
- Albright LJ, Wilson EM. Sub-lethal effects of several metallic salts-organic compounds combinations upon the heterotrophic microflora of a natural water. Water Res. 1974; 8: 101-105. Ref.: https://tinyurl.com/y94cbuds
- Anderson DM, Lively JS, Vaccaro RF. Copper complexation during spring phytoplankton blooms in coastal waters. Mar Res. 1984; 42: 677-695. Ref.: https://tinyurl.com/y7lmq5wq
- Couillard D, Chartier M, Mercier G. Bacterial leaching of heavy metals from aerobic sludge. Bioresour Technol. 1991; 36: 293-302. Ref.: https://tinyurl.com/y8a9tcfo
- Kobayashi N, Okamura H. Effects of heavy metals on sea urchin embryo development-Part 2 Interactive toxic effects of heavy metals in synthetic mine effluents. Chemosphere. 2005; 61: 1198-1203. Ref.: https://tinyurl.com/y92xphnv
- Ipeaiyeda AR, Onianwa PC. Sediment quality assessment and dispersion pattern of toxic metals from brewery effluent discharged into the Olosun river, Nigeria. Environ Earth Sci. 2016; 75: 325. Ref.: https://tinyurl.com/yb9ty5kw
- Aquino SF, Stuckey DC. Bioavailability and toxicity of metal nutrients during anaerobic digestion. Environ Eng. 2007; 133: 28-35. Ref.: https://tinyurl.com/ydbtwz6u
- Shi Y, Qi X, Gao Q. Removal of heavy metals by bacteria in bio-ceramsite and their toxicity to bacteria. Asian J Chem. 2015; 27: 2463-2467. Ref.: https://tinyurl.com/y7opseds
- Mendiguchía C, Moreno C, García-Vargas M. Separation of heavy metals in seawater by liquid membranes: preconcentration of copper. Sep Sci Technol. 2002; 37: 2337-2351. Ref.: https://tinyurl.com/y95hpbgo
- Ornaghi F, Ferrini S, Prati M, Giavini E. The protective effects of N-acetyl-L-cysteine against methyl mercury embryo toxicity in Mice. Toxicol Sci. 1993; 20: 437-445. Ref.: https://tinyurl.com/y9f8fryx
- Sato C, Leung SW, Schnoor JL. Toxic response of Nitrosomonas europaea to copper in inorganic medium and wastewater. Water Res. 1988; 22: 1117-1127. Ref.: https://tinyurl.com/y8mrco68
- McIver CJ, Tapsall JW. Cysteine requirements of naturally occurring cysteine auxotrophs of Escherichia coli. Pathology. 1987; 19: 361-363. Ref.: https://tinyurl.com/y8ryl9oh
- Sprague JB. Promising anti-pollutant: chelating agent NTA protects fish from copper and zinc. Nature. 1968; 220: 1345-1346. Ref.: https://tinyurl.com/yc998qxb
- Shaikh ZA, Blazka ME, Endo T. Metal transport in cells: cadmium uptake by rat hepatocytes and renal cortical epithelial cells. Environ Health Perspect. 1995; 103: 73-75. Ref.: https://tinyurl.com/y8o5fwqt
- Puls RW, Bohn HL. Sorption of cadmium, nickel, and zinc by kaolinite and montmorillonite suspensions1. Soil Sci Soc Am J. 1988; 52: 1289. Ref.: https://tinyurl.com/y9lqz7py
- Hall PL. Adsorption of water by homoionic exchange forms of Wyoming montmorillonite (SWy-1). Clays Clay Miner. 1989; 37: 355-363. Ref.: https://tinyurl.com/ycfxn6wj
- Leitao JH, Sa-Correia I. Effects of growth-inhibitory concentrations of copper on alginate biosynthesis in highly mucoid Pseudomonas aeruginosa. Microbiology. 1997; 143: 481-488. Ref.: https://tinyurl.com/ybhup42z
- Masuda G, Tomioka S, Uchida H, Hasegawa M. Bacteriostatic and bactericidal activities of selected Beta-Lactam antibiotics studied on agar plates. Antimicrob Agents Chemother. 1977; 11: 376-382. Ref.: https://tinyurl.com/yb6uqfvk
- Panda J, Sarkar P. Bioremediation of chromium by novel strains Enterobacter aerogenes T2 and Acinetobacter sp PD 12 S2. Environ Sci Pollut Res. 2011; 19: 1809-1817. Ref.: https://tinyurl.com/y996ak4h
- Sundar K, Sadiq M, Mukherjee A, Chandrasekaran N. Bioremoval of trivalent chromium using Bacillus biofilms through continuous flow reactor. Hazard Mater. 2011; 196: 44-51. Ref.: https://tinyurl.com/y7nu5yub
- Bhaskar RK. Pollutants induced cancer in experimental animals. Int J Sci Res. 2016; 5: 2221-2225. Ref.: https://tinyurl.com/ya7no6lg
- Cenci C, Morozzi G. Evaluation of the toxic effect of Cd2+ and Cd(CN)42− ions on the growth of mixed microbial population of activated sludges. Sci Total Environ. 1977; 7: 131-143. Ref.: https://tinyurl.com/y8xztqyp
- Ji G, Silver S. Reduction of arsenate to arsenite by the ArsC protein of the arsenic resistance operon of Staphylococcus aureus plasmid pI258. Proc Natl Acad Sci. 1992; 89: 9474-9478. Ref.: https://tinyurl.com/y73cdwkk
- Babai R. An Escherichia coli gene responsive to heavy metals. FEMS Microbiol Lett. 1998; 167: 107-111. Ref.: https://tinyurl.com/y7wgac4q
- Tempest DW, Hunter JR, Sykes J. Magnesium-limited growth of Aerobacter aerogenes in a chemostat. J Gen Microbiol. 1965; 39: 355-366. Ref.: https://tinyurl.com/y9ee43vx
- Tsai KP. Management of target algae by using copper-based algaecides: effects of algal cell density and sensitivity to copper. Water Air Soil Pollut. 2016; 227: 238. Ref.: https://tinyurl.com/yaz5pwf2
- Al-Masoudi WA, Faaz RA, Al-Asadi RH, Jabbar HS. Synthesis, antimicrobial activity and modelling studies of some new metal complexes of Schiff base derived from sulphonamide drug in vitro. Eur J Chem. 2016; 7: 102-106. Ref.: https://tinyurl.com/y7yewfx6
- Smit H, van der Goot H, Nauta WT, Timmerman H, de Bolster MW, et al. Mode of action of the copper(I) complex of 2,9-dimethyl-1,10-phenanthroline on Mycoplasma gallisepticum. Antimicrob Agents Chemother. 1981; 20: 455-462. Ref.: https://tinyurl.com/ycszynn2
- Harris CM, Patil HRH, Sinn E. Nitrogenous chelate complexes of transition metals. IV Pseudo-tetrahedral copper (II) complexes containing 2,2'-biquinolyl. Inorg Chem. 1967; 6: 1102-1105. Ref.: https://tinyurl.com/y7jvruh8
- Milacic V, Jiao P, Zhang B, Dou QP. Novel 8-hydroxylquinoline analogs induce copper-dependent proteasome inhibition and cell death in human breast cancer cells. Int J Oncol. 2009; 35: 1481-1491. Ref.: https://tinyurl.com/yd4nt7wv
- Naka K, Ando D, Chujo Y. Effect of substituent groups for formation of organic-metal hybrid nanowires by charge-transfer of tetrathiafulvalene derivatives with metal ion. Synth Met. 2009; 159: 931-934. Ref.: https://tinyurl.com/yah5oera
- Dipu S, Kumar AA, Thanga SG. Effect of chelating agents in phytoremediation of heavy metals. Remed J. 2012; 22: 133-146. Ref.: https://tinyurl.com/y76gajop
- Madoni P, Esteban G, Gorbi G. Acute toxicity of cadmium, copper, mercury, and zinc to ciliates from activated sludge plants. Bull Environ Contam Toxicol. 1992; 49: 900-905. Ref.: https://tinyurl.com/yd9fkowq
- Morgan-Sagastume F, Nielsen JL, Nielsen PH. Substrate-dependent denitrification of abundant probe-defined denitrifying bacteria in activated sludge. FEMS Microbiol Ecol. 2008; 66: 447-461. Ref.: https://tinyurl.com/yau2yrhk
- Gu Z, Aikebaier Y, Arefieva V, Mazirov M. Using microbiological leaching method to remove heavy metals from sludge. Eurasian J Soil Sci. 2017; 6: 51-51. Ref.: https://tinyurl.com/y8ptc6j8
- Abdulaziz A, Jasmin C, Sheeba VA, Gireeshkumar TR, Shanta N. Heavy metals pollution influence the community structure of Cyanobacteria in nutrient rich tropical estuary. Oceanogr. 2017; 3: 137. Ref.: https://tinyurl.com/yajlpdg3
- Chen J, Weimer PJ. Competition among three predominant ruminal cellulolytic bacteria in the absence or presence of non-cellulolytic bacteria. Microbiol. 2001; 147: 21-30. Ref.: https://tinyurl.com/ycqrydhb
- Vardy DW, Doering JA, Santore R, Ryan D, Giesy JP, et al. Assessment of Columbia river sediment toxicity to White Sturgeon: concentrations of metals in sediment, pore water and overlying water. J Environ Anal Toxicol. 2014; 5: 263. Ref.: https://tinyurl.com/ybnde9x5
- Meger SA. Polluted precipitation and the geochronology of mercury deposition in lake sediment of northern Minnesota. Water Air Soil Pollut. 1986; 30: 411-419. Ref.: https://tinyurl.com/yab68f4l
- Sarkar T, Hussain A. Photocytotoxicity of curcumin and its iron complex. Enzyme Eng. 2016; 5: 143. Ref.: https://tinyurl.com/ya3nzon6
- Ottow JCG. Evaluation of iron-reducing bacteria in soil and the physiological mechanism of iron-reduction in Aerobacter aerogenes. Z Allg Mikrobiol. 2007; 8: 441-443. Ref.: https://tinyurl.com/y8l8hxot
- Grass G. Iron Transport in Escherichia Coli: All has not been said and Done. Biometals. 2006; 19: 159-172. Ref.: https://tinyurl.com/y978dbfc
- Atieh MA, Ji Y, Kochkodan V. Metals in the environment: toxic metals removal. Bioinorg Chem Appl. 2017; 2017: 1-2. Ref.: https://tinyurl.com/ycx8ftlm
- Pongratz R, Heumann KG. Production of methylated mercury, lead, and cadmium by marine bacteria as a significant natural source for atmospheric heavy metals in polar regions. Chemosphere. 1999; 39: 89-102. Ref.: https://tinyurl.com/y7kpgjrp
- Maher WA. Determination of inorganic and methylated arsenic species in marine organisms and sediments. Anal Chim Acta. 1981; 126: 157-165. Ref.: https://tinyurl.com/y98qtw6t
- Farmer JG. Lead concentration profiles in lead-210 dated Lake Ontario sediment cores. Sci Total Environ. 1978; 10: 117-127. Ref.: https://tinyurl.com/yct52zds
- Kobza J. Soil and plant pollution by potentially toxic elements in Slovakia. Plant Soil Environ. 2018; 51: 243-248. Ref.: https://tinyurl.com/yb7lqkly
- Clarkson TW, Stockinger H. Recent advances in the toxicology of mercury with emphasis on the alkylmercurials. Crit Rev Toxicol. 1972; 1: 203-234. Ref.: https://tinyurl.com/ya4mbb6b
- Lambertsson L, Nilsson M. Organic material: the primary control on mercury methylation and ambient methyl mercury concentrations in estuarine sediments. Environ Sci Technol. 2006; 40: 1822-1829. Ref.: https://tinyurl.com/ybus2bxp
- Baralkiewicz D, Gramowska H, Gołdyn R. Distribution of total mercury and methyl mercury in water, sediment and fish from Swarze dzkie lake. Chem Ecol. 2006; 22: 59-64. Ref.: https://tinyurl.com/y8xhp5rp
- Wright DR, Hamilton RD. Release of methyl mercury from sediments: effects of mercury concentration, low temperature, and nutrient addition. Can J Fish Aquat Sci. 1982; 39: 1459-1466. Ref.: https://tinyurl.com/yd9ugadg
- Oswald CJ, Carey SK. Total and methyl mercury concentrations in sediment and water of a constructed wetland in the Athabasca Oil Sands Region. Environ Pollut. 2016; 213: 628-637. Ref.: https://tinyurl.com/ycfsq9wh
- Furukawa K, Tonomura K. Induction of metallic mercury-releasing enzyme in mercury-resistant pseudomonas. Agric Biol Chem. 1972; 36: 2441-2448. Ref.: https://tinyurl.com/y8puseek
- Furukawa K, Tonomura K. Enzyme system involved in the decomposition of phenyl mercuric acetate by mercury-resistant pseudomonas. Agric Biol Chem. 1971; 35: 604-610. Ref.: https://tinyurl.com/y7z2uaau
- Matsumura F, Gotoh Y, Boush GM. Phenylmercuric acetate: metabolic conversion by microorganisms. Science. 1971; 173: 49-51. Ref.: https://tinyurl.com/yamjhjkw
- Graham AM, Bullock AL, Maizel AC, Elias DA, Gilmour CC. Detailed assessment of the kinetics of Hg-cell association, Hg methylation, and methylmercury degradation in several Desulfovibrio species. Appl Environ Microbiol. 2012; 78: 7337-7346. Ref.: https://tinyurl.com/y9f52eeg
- Alekhin YV, Zagrtdenov NR, Mukhamadiyarova RV. Hg0(liq)-Hg0(solution) equilibrium and solubility of elementary mercury in water. Moscow Univ Geol Bull. 2011; 66: 439-441. Ref.: https://tinyurl.com/y8725ndh
- Krul J. Some factors affecting floc formation by Zoogloea ramigera, strain I-16-M. Water Res. 1977; 11: 51-56. Ref.: https://tinyurl.com/yctttc7l
- Bitton G, Freihofer V. Influence of extracellular polysaccharides on the toxicity of copper and cadmium toward Klebsiella aerogenes. Microb Ecol. 1977; 4: 119-125. Ref.: https://tinyurl.com/ycts6oog
- Brandt KK, Petersen A, Holm PE, Nybroe O. Decreased abundance and diversity of culturable Pseudomonas spp. populations with increasing copper exposure in the sugar beet rhizosphere. FEMS Microb Ecol. 2006; 56: 281-291. Ref.: https://tinyurl.com/ydx6oxn9
- Tornabene TG, Edwards HW. Microbial uptake of lead. Science. 1972; 176: 1334-1335. Ref.: https://tinyurl.com/y9v7m2hj
- Kim SJ. Research papers: Estimation of active nitrosomonas and nitrobacter concentrations in activated sludge using nitrogenous oxygen uptake rate. Environ Eng Res. 2004; 9: 130-142. Ref.: https://tinyurl.com/ycc43336
- Yu R, Lai B, Vogt S, Chandran K. Elemental profiling of single bacterial cells as a function of copper exposure and growth phase. PLoS ONE. 2011; 6: e21255. Ref.: https://tinyurl.com/y856j8r2
- TyagiRD.Microbialleachingof metals frommunicipal sludge: Effects of sludge solids concentration. Process Biochem. 1992; 27: 89-96. Ref.: https://tinyurl.com/ydf6lgkg
- Komura I, Funaba T, Izaki K. Mechanism of mercuric chloride resistance in microorganisms: II. NADPH-dependent reduction of mercuric chloride and vaporization of mercury from mercuric chloride by a multiple drug resistant strain of Escherichia coli. J Biochem. 1971; 70: 895-901. Ref.: https://tinyurl.com/y92wdb8x
- Rochelle PA, Fry JC, Day MJ. Factors affecting conjugal transfer of plasmids encoding mercury resistance from pure cultures and mixed natural suspensions of Epilithic Bacteria. Microbiol. 1989; 135: 409-424. Ref.: https://tinyurl.com/y9dgtbvu
- Guha C, Mookerjee A. RNA synthesis and degradation during preferential inhibition of protein synthesis by cobalt chloride in Escherichia coli K-12. Mol Biol Rep. 1981; 7: 217-220. Ref.: https://tinyurl.com/y9g5sjge
- Gotz F, Zabielski J, Philipson L, Lindberg M. DNA homology between the arsenate resistance plasmid pSX267 from Staphylococcus xylosus and the penicillinase plasmid pI258 from Staphylococcus aureus. Plasmid. 1983; 9: 126-137. Ref.: https://tinyurl.com/ybbt4uf7
- Diels L, Sadouk A, Mergeay M. Large plasmids governing multiple resistances to heavy metals: A genetic approach. Toxicol Environ Chem.1989; 23: 79-89. Ref.: https://tinyurl.com/yaqcrmoz
- Berger NA, Kociolek K, Pitha J. Steric factors in lymphocyte stimulation by organomercurials. Biochem Biophys Res Commun. 1979; 86: 1234-1240. Ref.: https://tinyurl.com/y76h4nuk
- Schwager S, Lumjiaktase P, Stöckli M, Weisskopf L, Eberl L. The genetic basis of cadmium resistance of Burkholderia cenocepacia. Environ Microbiol Rep. 2012; 4: 562-568. Ref.: https://tinyurl.com/yacvdrzs
- Gillis P. Investigating a potential mechanism of Cd resistance in Chironomus riparius larvae using kinetic analysis of calcium and cadmium uptake. Aquat. Toxicol. 2008; 89: 180-187. Ref.: https://tinyurl.com/y8xwjr5g
- Fujiwara K, Iwamoto M, Toda S, Fuwa K. Characteristics of Escherichia coli B resistant to cobaltous ion. Agric Biol Chem. 1977; 41: 313-322. Ref.: https://tinyurl.com/yanhrmjl
- Grabow WOK, van Zyl M, Prozesky OW. Behaviour in conventional sewage purification processes of coliform bacteria with transferable or non-transferable drug-resistance. Water Res. 1976; 10: 717-723. Ref.: https://tinyurl.com/y7anmj4m
- Varma MM, Thomas WA, Prasad C. Resistance to inorganic salts and antibiotics among sewage-borne enterobacteriaceae and achromobacteriaceae. J Appl Bacteriol. 1976; 41: 347-349. Ref.: https://tinyurl.com/y8hvo6f6
- Sinegani AAS, Younessi N. Antibiotic resistance of bacteria isolated from heavy metal-polluted soils with different land uses. J Glob Antimicrob Resist. 2017; 10: 247-255. Ref.: https://tinyurl.com/y8xsd7g3
- Nakahara H, Ishikawa T, Sarai Y, Kondo I, Kozukue H, et al. Mercury resistance and R Plasmids in Escherichia coli isolated from clinical lesions in Japan. Antimicrob Agents Chemother. 1977; 11: 999-1003. Ref.: https://tinyurl.com/ycaok7w2
- Baquero F. Low-level antibacterial resistance: a gateway to clinical resistance. Drug Resist Update. 2001; 4: 93-105. Ref.: https://tinyurl.com/ybg4xhwr
- Yamina B, Tahar B, Laure FM. Isolation and screening of heavy metal resistant bacteria from wastewater: a study of heavy metal co-resistance and antibiotics resistance. Water Sci Technol. 2012; 66: 2041. Ref.: https://tinyurl.com/y86gs5ok
- Wales A, Davies R. Co-selection of resistance to antibiotics, biocides and heavy metals, and its relevance to food borne pathogens. Antibiotics. 2015; 4: 567-604. Ref.: https://tinyurl.com/ya7kogdh
- Altug G, Balkis N. Levels of some toxic elements and frequency of bacterial heavy metal resistance in sediment and sea water. Environ Monit Assess. 2008; 149: 61-69. Ref.: https://tinyurl.com/y86tm8gz
- Guo T, Baasner J. Technical Note: Using FIMS to determine mercury content in sewage sludge, sediment and soil samples. J Autom Chem. 1996; 18: 221-223. Ref.: https://tinyurl.com/yanwhpfh
- Hart M. Diversity amongst Bacillus merA genes amplified from mercury resistant isolates and directly from mercury polluted soil. FEMS Microbiol Ecol. 1998; 27: 73-84. Ref.: https://tinyurl.com/ycu33hfr
- Søgaard P. Resistance types in citrobacter freund II occurrence and resistance to ampicillin, carbenicillin, cephalothin and mecillinam. Transfer of ampicillin resistance. Acta Pathol Microbiol Scand B. 2009; 27: 79-83. Ref.: https://tinyurl.com/y7t5cfjk